Pulsed Power for Fundamental Plasma Physics and Laboratory Astrophysics
High Energy Density (HED) experiments use intense lasers or powerful pulses of electrical current to heat initially solid targets to form dense, hot plasmas which mimic the conditions found in extreme astrophysical phenomena, such as the Weibel instability in collisionless shocks, or jets from young stars. However, these extreme conditions can only be sustained on short timescales, often much smaller than the characteristic hydrodynamic or Alfvénic times in the plasma. Many interesting and important plasma instabilities grow on these characteristic timescales, and so they may not be able to fully develop in short-lived HED plasmas.
Pulsed-power generators drive plasmas over much longer timescales than lasers, and at a low cost per Joule-on-target. Existing pulsed-power generators are optimized for rapidly rising current pulses (100 – 200 ns) and high voltages (~MV), which are necessary to drive imploding loads with changing inductance. However, non-imploding loads can be driven by lower voltages, and without pulse-compression. This allows for cheaper, more compact generators, which also drive plasmas on the long timescales necessary for comparison with theory and astrophysical phenomena [1].
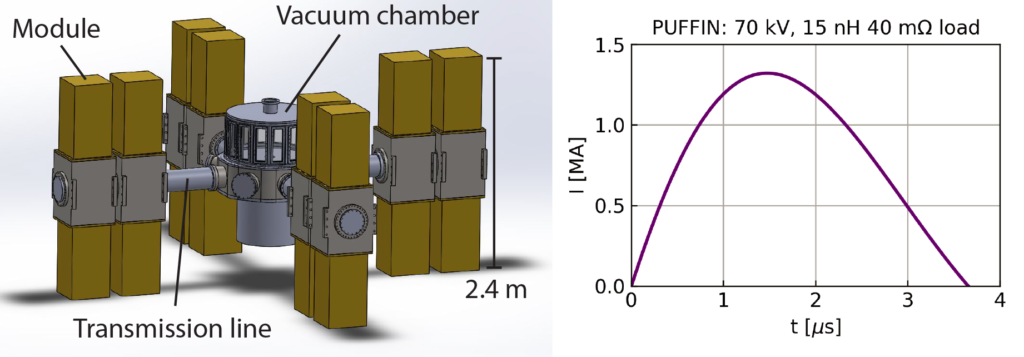
PUFFIN will use eight LTD-5 stages, arranged in four branches of two modules, to produce a 1.5 MA peak current with a 1.5 microsecond rise time, depending on the load inductance. PUFFIN is very versatile, and can drive a range of loads in different geometries to produce jets, magnetic reconnection, magnetized heat flow, shocks and magnetized instabilities. In particular, PUFFIN is ideally suited for experiments with MHD turbulence, a unique capability of this device which sets it apart from other experimental facilities (see below).
PUFFIN is modular and upgradeable: adding additional stages will drive either higher currents and magnetic fields, or higher voltages for more inductive loads. It will directly involve undergraduate and graduate students in construction and in experiments, providing valuable hands-on training. Construction of PUFFIN will start at the Plasma Science and Fusion Center at MIT in 2021.
[1] Lebedev, S. V., A. Frank, and D. D. Ryutov. “Exploring Astrophysics-Relevant Magnetohydrodynamics with Pulsed-Power Laboratory Facilities.” Reviews of Modern Physics 91, no. 2 (April 25, 2019): 025002. https://doi.org/10.1103/RevModPhys.91.025002.
Magnetohydrodynamic Turbulence
Turbulence is a fundamental process in all fluids, including plasmas. It dissipates large scale fluid motions through a cascade to ever smaller scales, until viscosity dominates and dissipates the kinetic energy as heat. In a plasma, magnetic fields couple to the charged particles, breaking the symmetry and creating anisotropic, fully three-dimensional turbulence. In MHD, the basic building blocks of turbulence are magnetic flux tubes, which interact, creating current sheets which in turn drive flux-tube merging through magnetic reconnection.
In a plasma, the familiar Reynolds number , has a magnetic counterpart
, where
is the magnetic diffusivity. Both must be large to sustain MHD turbulence, requiring a high-energy-density (HED) state with high
and high
. Such plasmas can be created by high-powered lasers or pulsed-power driven currents. Pulsed-power creates long lasting, inherently magnetised plasmas, with an astrophysically-relevant equipartition between the magnetic, thermal and kinetic energies. This is in contrast to long-lived magnetic confinement plasmas, dominated by magnetic pressure, or short-lived laser-driven plasmas, dominated by kinetic and thermal pressures.
Existing laser-driven experiments create a turbulent plasma which then rapidly decays, without any further injection of energy at the outer scale. Energy injection can be sustained using pulsed-power generators, which can drive the plasma over many Alfvénic timescales. The PUFFIN current pulse is times longer than existing pulsed-power facilities, and combined with the astrophysically relevant equipartition between the energy components, PUFFIN provides a unique and ideal driver for MHD turbulence experiments.
Platforms for Generating Turbulence
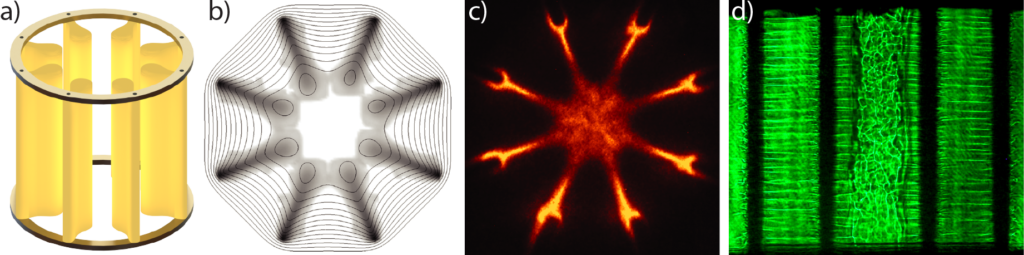
An imploding wire array initially produces plasma around each wire with an O-point magnetic field structure.The global magnetic field around all the wires then sweeps these plasmas and their magnetic fields inwards, creating a flux-tube-merging geometry reminiscent of MHD turbulence. By using a low-Z element like carbon, we minimize radiative losses, keeping the plasma hot and the Reynolds numbers high. Evidence for turbulence in this plasma comes from laser imaging diagnostics, which show the formation of structures on all resolvable spatial scales.
Another platform uses shear flows, produced by modifying our reconnection platform to include obstacles in the colliding flows. This velocity shear will generate a magnetized Kelvin-Helmholtz instability, which tangles the magnetic field lines and drives turbulence. Shear flows are a common technique for rapidly generating turbulence in numerical simulations, and preliminary experiments suggest it is also a powerful technique in the laboratory.
New Diagnostics for Turbulence
We recently designed and tested a new diagnostic at the MAGPIE group, an Imaging Refractometer, which uses cylindrical and spherical lenses to directly image the spectrum of deflection angles in a probing laser beam, rather than inferring it from Fourier transforms of pixel-size-limited images [2]. This spectrum of deflection angles can be linked to the spectrum of plasma density fluctuations, and we have already achieved a dynamic range of over two orders of magnitude. Notably, we observe non-Gaussian features in the spectrum of deflection angles, which we have linked to intermittency in the plasma turbulence using numerical simulations and synthetic diagnostics.
In addition to this diagnostic, we can use spatially resolved Thomson scattering to make local measurements of the velocity, density and temperature fluctuations, and use Faraday Rotation Imaging to study the magnetic field fluctuations. The combination of these advanced diagnostic, along with the unique platforms for generating MHD turbulence, will provide high quality data for detailed comparisons with theory and simulations.
[1] Martin, M. R., C. E. Seyler, and J. B. Greenly. “The Role of Magnetic Field in the Transition to Streaming Ablation in Wire Arrays.” Physics of Plasmas 17, no. 5 (2010): 052706. https://doi.org/10.1063/1.3392288.
[2] Hare, J. D., G. C. Burdiak, S. Merlini, J. P. Chittenden, T. Clayson, A. J. Crilly, J. W. D. Halliday, et al. “An Imaging Refractometer for Density Fluctuation Measurements in High Energy Density Plasmas.” ArXiv:2007.04682 [Physics]. http://arxiv.org/abs/2007.04682.
Magnetic Reconnection
Magnetic reconnection is a ubiquitous and important process throughout the Universe. It explosively reconfigures the topology of magnetic field lines, and enables the rapid dissipation of magnetic energy, heating and accelerating the plasma. This process has been studied in both space and laboratory experiments, including the collisionless regime relevant to the solar wind. In recent years, high energy density experiments driven by lasers or pulsed-power have further expanded the plasmas in which we can study reconnection, and in this white paper I will discuss new experiments to explore new regimes of magnetic reconnection
Reconnection in collisional plasmas has received relatively little experimental interest, despite its importance to many astrophysical objects, such as black hole accretion disks, or the opaque convection zone of the Sun. In contrast to the solar wind, it is difficult to make either in-situ or remote sensing measurements of reconnection in these remote environments, which underscores the need for detailed experimental work to validate theories and numerical simulations. These collisional plasmas can be created using a pulsed-power driven platform [1], which I will use to study three key aspects of magnetic reconnection: the formation of the plasmoid instability, the development of three-dimensional instabilities, and the importance of radiative cooling.
The plasmoid instability
The plasmoid instability is a tearing of the reconnecting current sheet, which leads to very rapid reconnection and dissipation of the magnetic energy. It is believed to play a ubiquitous role in setting the rate of reconnection in many astrophysical plasmas. In MHD reconnection, the plasmoid instability is expected to occur at Lundquist numbers greater than , which is very challenging to achieve in laboratory plasmas.
However, two-fluid effects lead to a semi-collisional regime of this instability, in which plasmoids can form at much more modest Lundquist numbers [2], including under the conditions found within the solar corona.
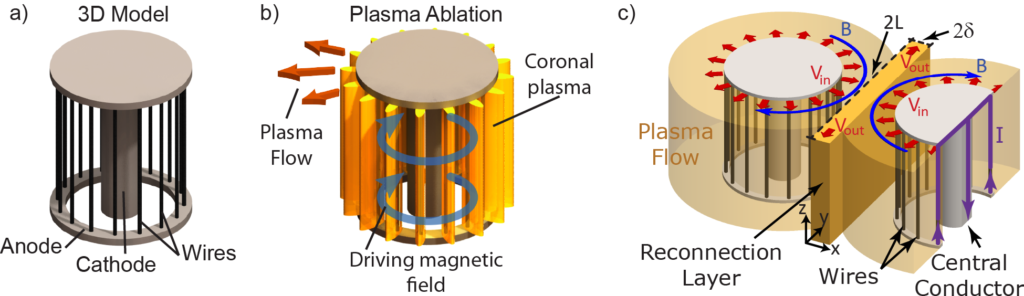
I observed these semi-collisional plasmoids experimentally for the first time [3]. Although the precise physics may differ quantitatively from purely MHD plasmas, these observations offer a qualitative glimpse into plasmoid dynamics in otherwise inaccessible regimes. I will build on these observations by addressing open questions about the fully non-linear state of the instability, which includes plasmoid merging and a transition to turbulent reconnection. These questions are relevant to outstanding problems in reconnection, such as the onset problem, and the partition of energy. PUFFIN will be able to reach this non-linear state for the first time, because it can drive the plasma for times longer than our previous experiments.
Three-dimensional instabilities
Although magnetic reconnection is often modeled and studied in two-dimensions, any realistic plasma will have three-dimensional dynamics. Indeed, astrophysical observations suggests that reconnection is volume-filling, implying the presence of turbulence which broadens the reconnection layer. This turbulence may be produced by kink instabilities in the current-carrying plasmoids, which in numerical simulations grow to fill the entire simulation volume [4]. Such instabilities can also lead to self-organization of the plasma, as magnetic energy is driven by an inverse cascade to larger length scales. Observing the full development of this kink instability in the laboratory will require the long drive times uniquely provided by PUFFIN.
Radiative reconnection
In extreme astrophysical environments, the radiative losses from a plasma can form a significant part of the energy partition in the reconnection process. Radiative reconnection is an almost entirely unexplored frontier of reconnection physics, which contains a wide variety of rich fundamental processes. For example, simple models of radiative reconnection predict a radiative cooling instability which leads to a dramatic collapse of the reconnection layer [5]. Preliminary experiments on MAGPIE have demonstrated this effect in the laboratory [6], in which the reconnection layer rapidly cooled and densified. I am working with researchers at Sandia National Labs on experiments for the Z Fundamental Science Program, which will enable us to study radiative reconnection on the Z-machine, the largest pulsed-power driver in the world (20-30 MA, 100-1000 ns).
[1] Hare, J. D., L. G. Suttle, S. V. Lebedev, N. F. Loureiro, A. Ciardi, J.P. Chittenden, T. Clayson, et al. “An Experimental Platform for Pulsed-Power Driven Magnetic Reconnection.” Physics of Plasmas 25 (2018): 055703. https://doi.org/10.1063/1.5016280.
[2] Loureiro, N F, and D A Uzdensky. “Magnetic Reconnection: From the Sweet–Parker Model to Stochastic Plasmoid Chains.” Plasma Physics and Controlled Fusion 58 (2015): 014021. https://doi.org/10.1088/0741-3335/58/1/014021.
[3] Hare, J D, L Suttle, S V Lebedev, N F Loureiro, A Ciardi, G C Burdiak, J P Chittenden, et al. “Anomalous Heating and Plasmoid Formation in a Driven Magnetic Reconnection Experiment.” Physical Review Letters 118 (2017): 085001. https://doi.org/10.1103/PhysRevLett.118.085001.
[4] Lapenta, G., and L. Bettarini. “Spontaneous Transition to a Fast 3D Turbulent Reconnection Regime.” EPL (Europhysics Letters) 93, no. 6 (March 1, 2011): 65001. https://doi.org/10.1209/0295-5075/93/65001.
[5]Uzdensky, Dmitri A., and Jonathan C. McKinney. “Magnetic Reconnection with Radiative Cooling. I. Optically Thin Regime.” Physics of Plasmas 18 (2011): 042105. https://doi.org/10.1063/1.3571602.
[6] Suttle, L. G., J. D. Hare, S. V. Lebedev, A. Ciardi, N. F. Loureiro, G. C. Burdiak, J. P. Chittenden, et al. “Ion Heating and Magnetic Flux Pile-up in a Magnetic Reconnection Experiment with Super-Alfvénic Plasma Inflows.” Physics of Plasmas 25 (2018): 042108. https://doi.org/10.1063/1.5023664.